Phenotypic Trait Variation in Populations of a Global Invader Mayweed Chamomile (Anthemis cotula): Implications for Weed Management
- 1Department of Entomology, Plant Pathology and Nematology, University of Idaho, Moscow, ID, United States
- 2Department of Crop and Soil Sciences, Washington State University, Pullman, WA, United States
- 3Statistical Programs, College of Agricultural and Life Sciences, University of Idaho, Moscow, ID, United States
Mayweed chamomile (Anthemis cotula L.) is an annual crop weed that has become a substantial impediment to diversify traditional wheat-based cropping systems such as in the Pacific Northwest (PNW), USA. Some of the broadleaf rotational crops are vulnerable to the weed as they are less competitive or lack compatible herbicides for A. cotula management. Although A. cotula has been present in the PNW for more than a century, traits that contribute to invasiveness and how these vary among the weed populations have not been investigated. We assessed trait variation with a common garden greenhouse experiment by comparing 19 A. cotula populations from the PNW and Kashmir Valley, India. Seeds from individual plants from each population were used to grow plants through their senescence. We measured phenological (e.g., flowering duration), morphological (e.g., plant biomass), reproductive fitness (e.g., number of flower heads), and physiological (floral scent VOCs: volatile organic compounds) traits on individual plants and analyzed the data using mixed-effects models. We found high inter-population variation in most of the traits measured, but the variation was not associated with the geographical distance. Seedling emergence within 30 days of planting ranged from 2 to 49% for PNW populations to 55 to 72% for Indian populations. Flowering duration ranged from 2 to 3 months among populations. Other traits such as initial and final flowering dates, the total number of flower heads, and floral scent VOCs also differed among populations. These trait patterns may indicate a localized adaptation of A. cotula populations to environmental or management regimes, variation arising from anthropogenic or natural dispersal, multiple introductions, genetic drift, or combinations of these. Regardless of the processes, the trait variation suggests that weed management plan for A. cotula may need to be tailored to specific locations instead of a uniformly adopted plan across the region.
Introduction
Mayweed chamomile (Anthemis cotula L.) is an annual, bushy, ill-scented globally invasive weed (Kay, 1971; Adhikari et al., 2020a) that is especially problematic under the Mediterranean and Mediterranean-like climates such as in the Pacific Northwest (PNW), USA (Lyon et al., 2017) and the Kashmir valley, India (Shah et al., 2008, 2009). It prefers moist soils and commonly found in poorly drained areas, disturbed lands, field edges, roadsides, and in crop fields (Kay, 1971). It is native to Eurasia in regions with Mediterranean climates. In the modern era, it has been introduced, presumably as a contaminant of crop seed and other plant materials, and distributed globally (Kay, 1971; Mack and Erneberg, 2002; CABI, 2018). In Europe, it is considered as archaeophyte (anciently introduced plant species) in some countries, whereas outside this continent the species was first reported in South America (1816), followed by Africa (1838), North America (1841), Australia (1873), Antarctica (1903), and Asia (1933) (Global Biodiversity Information Facility, 2018) [details of global distribution are reviewed and reported in Adhikari et al. (2020a)].
Globally, there has been limited study (if any) of the ecological and economic threats of A. cotula to the local ecosystems. It is aggressively weedy in many croplands and pastures, reducing crop yields and forage quality (Adhikari et al., 2020a). In cereal-based cropping systems worldwide, climate change is motivating producers to diversify their operations by incorporating fall- and spring-sown pulses, canola, and cover crops (Eigenbrode et al., 2013; O'Leary et al., 2018). In the Pacific Northwest (PNW), USA, A. cotula was first reported in 1877 and has been a problematic weed, especially in cereal-based systems, reducing crop yield and contaminating harvests (Smitchger et al., 2012; Adhikari et al., 2020a). In the PNW, specifics of diversification differ with the climatic zone (Douglas et al., 1992; Karimi et al., 2018). Many of these crops are either less competitive with A. cotula than the cereal crops or prevent the use of effective herbicides for its management (Lyon et al., 2017). As a result, although it has been present for decades in the PNW that is historically known for cereal productions and exports, anecdotal accounts from local producers indicate that A. cotula is one of the significant barriers to the adoption of new crops such as cover crops and broadleaf rotational crops in the region. Despite these issues, there has been no systematic assessment of the biology of A. cotula in the PNW or elsewhere and whether its populations differ in phenotypic traits that contribute to its invasiveness and weediness.
Reproductive traits are critical for invasive populations to maintain their fitness and increase their invasiveness. In the PNW, A. cotula blooms from May to November, producing up to 200 conspicuous and attractive flower heads with white rays and yellow disc florets. Like many other asters (Rollin et al., 2016; Miller et al., 2018), it is self-incompatible (Kay, 1971), and the flowers are visited by a diverse suite of insects (Adhikari et al., 2020a). The traits such as abundant flower heads and long flowering periods of A. cotula might help attract and compete for pollinators maximizing its seed production. Each A. cotula can produce thousands of small (length ≤ 2 mm and weight ≤ 0.5 mg) seeds that are primarily dispersed by human activities (Shimono and Konuma, 2008; CABI, 2018). Whether germinate immediately or remain dormant for up to 25 years in the soil (Darlington, 1931), the capacity of A. cotula seeds to germinate facultatively (Ilnicki and Johnson, 1959) should facilitate the spread and persistence of A. cotula infestations across landscapes.
Within and among field agricultural management practices that differ across farms and climatic zones in the PNW could cause genetic trait variation in A. cotula, facilitating adaptation to formerly hostile climate niches of the species. Such local adaptation facilitates survival or avoidance of human and climatic selection pressures by maximizing plant fitness (Joshi et al., 2001; Baythavong, 2011; Lemke et al., 2015) and buffering the weed against short-and long-term environmental (e.g., climate, disturbance) fluctuations (Peterson et al., 2019). Alternatively, although A. cotula seeds do not have a specialized mechanism for long-distance dispersal, human activity could counteract local adaptation by moving seeds (e.g., via uncleaned crop seeds and farm equipment) of A. cotula randomly among infested and non-infested sites in the landscape, homogenizing populations. Pollen-mediated gene flow also homogenizes the populations at local scales (Hokanson et al., 1997; Bai et al., 2014; Ohadi et al., 2017). Human activity could drive or accentuate localized variation if movements are non-random and caused by land ownership and farm-specific management practices.
Invasion by A. cotula is part of a global pattern of unprecedented species invasion. Invasive species have displaced native species, negatively affected biodiversity, altered ecosystem structure and function, and disrupted natural and agricultural landscapes, causing severe ecological and economic impacts worldwide (Vilà et al., 2011; Van Kleunen et al., 2015; Pyšek et al., 2020). Considerable economic effects occur in cropping systems where invasive weeds cause an estimated annual cost of $1 billion in African smallholder farms (Pratt et al., 2017), $1.4 billion in the UK, $2.4 billion in Australia, and $27 billion in the USA (Pimentel et al., 2001, 2005; Oerke, 2006). Cropping systems are particularly vulnerable to plant invasion because intensive farming practices have fragmented, simplified, or disturbed landscapes, facilitating invasive weeds while contributing to the loss of native species (Tilman et al., 2011; Chaudhary et al., 2016; Adhikari et al., 2019). Climate change exacerbates the adverse effects of invasions (Bradley et al., 2010). Climate change includes the direct impacts of climate change on weed and crop interactions (Bradley et al., 2010; Matzrafi et al., 2019; Ziska et al., 2019) and indirect effects of changes in cropping systems in response to climate change (Chongtham et al., 2019; Weisberger et al., 2019). Invasive agricultural weeds like A. cotula, with wide distributions and long invasion histories, can become more difficult to manage under these circumstances (Adhikari et al., 2020b; Sharma et al., 2020), necessitating additional study to understand invasion drivers, future invasion potential, and avenues to improve management. Knowledge of the phenotypic and genotypic variation in invasive weed populations can help understand their invasiveness (Richards et al., 2006) and guide optimum management to mitigate their negative impacts on ecosystems (Sterling et al., 2004; Vander Zanden et al., 2010).
Anthemis cotula is a model species for globally invasive annual agricultural weeds. Critical for understanding these invasions is the delineation of the variability of invasive species throughout invaded landscapes. Genetic and phenotypic variability or uniformity has implications for ongoing invasiveness and current management practices. To understand the invasion ecology of A. cotula in the PNW, we investigated the interpopulation variation of its key performance and reproductive traits using a common garden greenhouse experiment. We collected A. cotula seeds from farms in the inland PNW spanning different precipitation zones (Supplementary Table 1) and compared seedling emergence, number of branches, plant height, plant volume, initial date of budding, initial and final date of flowering, anthesis, number of flower heads, flower size, number of florets, flowering duration, plant life, biomass production, and floral scent profiles among populations. To compare with PNW populations, we also included populations from Kashmir Valley, India (Figure 1), where A. cotula was first reported in 1972 (Stewart, 1972) and widely occurs in disturbed habitats of forests, rangelands, wastelands, and most recently it is invading the fringes of agricultural lands (Shah et al., 2008, 2009; M. Shah, Pers. Comm.). Anthemis cotula in these two regions, that have similar Mediterranean-like climates as in its native range, is considered as a problematic weed. Most of the studies (though they are limited) in A. cotula regarding its seed germination, competition with crops, plasticity, and abiotic and biotic interactions have been conducted either in PNW or in Kashmir Valley (see Adhikari et al., 2020a). However, none of these studies have focused on phenotypic trait comparisons among populations. We asked: (1) How do phenotypic traits vary among A. cotula populations? (2) Does the variation increase with geographical distance? (3) What processes most likely account for the trait variation? (4) Does this variation have implications for weed management?
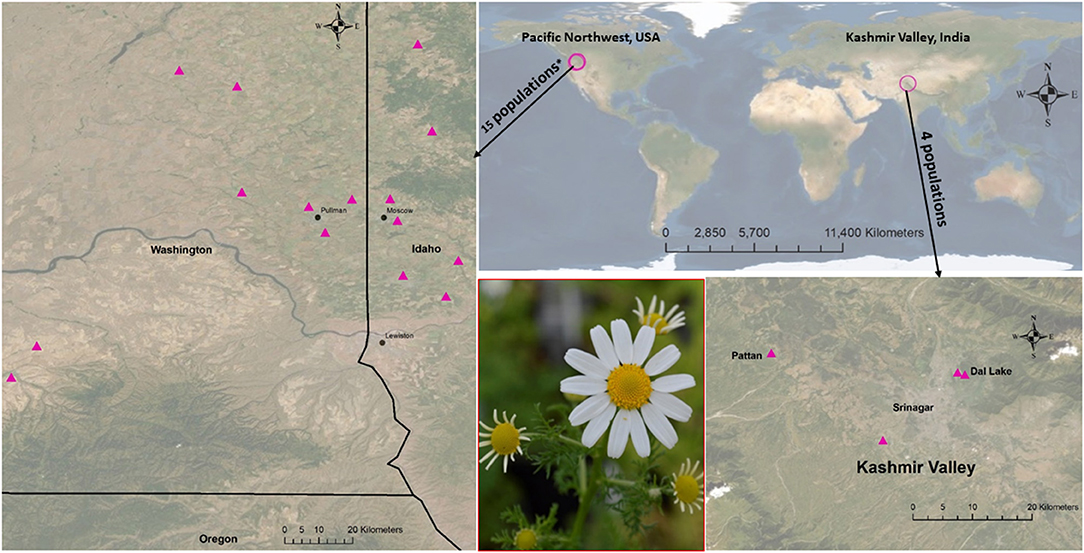
Figure 1. Maps showing 19 population origins (see Supplementary Table 1 for each site's climate and geographical details). There were 15 populations from the Pacific Northwest* (11 PNW1 + 4 PNW2) and four from Kashmir Valley, India.
Materials and Methods
This paper is a companion to Adhikari et al. (2021), which used a common greenhouse experiment to assess intrapopulation trait variation for PNW populations of A. cotula. Here, further data were collected from that experiment to include additional populations from the PNW and from India to evaluate interpopulation trait variation for the weed within and among regions. Therefore, the methods are similar to those in Adhikari et al. (2021).
Seed Collection
Seeds of 22 A. cotula populations were collected in two ways. In one method, we visited 11 known A. cotula on-farm infestation sites (i.e., populations) in the PNW across different precipitation or agroecological zones (see Supplementary Table 1 for site details) during 2018 and collected seeds from six different individuals (hereafter, PNW1: Foothill road, Genesee, Kambitsch, Palouse, Parker Farm, Potlatch, Spillman Farm, St. John, Tensed, Thornton, and Troy). Seeds from each individual were kept separate, constituting a half-sib family. For the other samples, we used A. cotula seeds collected and sent by local producers from PNW (four populations, hereafter, PNW2: Colfax, Cook Farm, Dayton 1, and Dayton 2) as well as seeds provided by scientific collaborators collected in the eastern USA (three populations: Illinois, Michigan, and New York) and Kashmir valley, India (four populations, hereafter Indian: Indian 1, Indian 2, Indian 3, and Indian 4). The seeds from producers and collaborators were not separated by source plant. All seeds were collected from A. cotula infesting crop fields except for seeds of Indian origin, which were collected from uncultivated lands.
Greenhouse Common Garden Experiment
To measure phenotypic traits among 19 populations, an experiment was conducted from February 27 to August 25, 2019. Twenty seeds from each plant from the 11 populations in which seed maternity was known (66 individuals) and the seeds of each of the other 11 populations were planted (n = 5) for a total of 385 pots (13 × 13 × 13.5 cm), to measure inter-population variation in phenotypic traits. The 2.3 L pots (spaced at 10 cm) filled with a commercial greenhouse soil mix (PRO-MIX BX containing 75 to 80% Canadian sphagnum peat moss along with perlite and vermiculite) were distributed on the greenhouse bench set at 15-h photoperiod of sunlight and supplemental artificial light (photosynthetic photon flux = 595 μmol/s) in a randomized complete block design with five blocks (average temperature = 22.9 ± 0.26°C and humidity = 43.7 ± 9.5% (mean ± SE). The space between blocks was maintained at 30 cm. Pots were regularly watered as needed and not fertilized. Two populations from the eastern USA (from Illinois and Michigan) did not germinate by 30 days, and another (from New York) was determined to be scentless chamomile or false mayweed [Tripleurospermum maritimum (L.) W.D.J. Koch] and were excluded from the study. Maps of the seed collecting sites of the remaining 19 populations are shown in Figure 1, and the details including GPS locations, elevation, and edaphic and climatic variables are provided in supplementary (Supplementary Table 1) materials (additional details in Adhikari et al., 2021).
Phenotypic Traits
We collected data on 15 phenotypic traits from each plant in the study. There were four phenotypic categories: (a) phenological (7): percent seedling emergence in first 30 days, total days taken to first budding (time spent on vegetative growth), days from budding to flowering (days in anthesis), the initial date of flowering, the final date of flowering (days), flowering duration (days), and plant life (days); (b) morphological (4): plant height (cm), number of branches, plant volume (πd2/4 × h, d = average plant diameter in cm and h = plant height in cm) at first flowering, and aboveground dry biomass (g) at harvest; (c) reproductive (3): total number of flower heads and size and number of ray florets of first or terminal flower; and (d) physiological (1): floral scent profiles. All traits were measured on every plant in the study except for flower size, number of ray florets, and floral scent characterization. Flower size and number of ray florets were measured on a sample of five blooming plants per population, and floral scent characterization were measured on a sample of 4 to 9 blooming plants per population.
Floral Scent Analysis
Due to the potentially important, unique, and prominent scent of A. cotula flowers (aka stinking chamomile) as well as time and resource constraints, we focused on floral scents only, but other physiological traits can also be associated with the invasiveness. In addition to other floral traits (Rowe et al., 2020), floral scent is also a key trait for pollinator-dependent plants' fitness (Majetic et al., 2009; Schiestl, 2015). As an obligate out-crosser and a generalist species (Kay, 1971; Adhikari et al., 2020a), A. cotula floral scents could attract a wide range of pollinators and maximize seed production and increase its invasiveness. For the floral scent characterization of A. cotula, we chose 11 populations (seven from PNW1, two from PNW2, and two from Kashmir valley, India) with at least four plants, each with five flowers blooming at the time of volatile collection. Solid phase micro-extraction (“SPME”: field sampler with 100-μm polydimethylsiloxane coated fiber) was used to collect volatile organic compounds (VOCs) from 4 to 9 available individuals from each population, and VOC profiles were compared among all 11 populations. Five live flowers, still attached to plants, were inserted into a 90 mL glass tube, and cotton was pushed in around the stems at the base of the tube to contain the airspace (Supplementary Figure 1). SPME fibers were then inserted through a 1 mm hole of a septum (PTFE silicon septum; 10 mm diameter) in the top of the tube and exposed for an hour. The fibers were then retracted for further analysis in gas chromatography/mass spectrometry (GC/MS; Agilent 7890A gas chromatograph/5973 Mass Selective Detector); helium was used as the carrier gas, and the injector temperature was held at 250°C. Volatile organic compounds were provisionally identified based on match (≥80%) between their spectra and National Institute of Standards and Technology-11 spectral library linked to Agilent Mass Hunter software. Abundance (total chromatogram m/z; mass-to-charge ratio), richness (expressed as the number of compounds), Simpson's diversity (Simpson, 1949), and Pielou's evenness (Pielou, 1966) of VOCs are presented here (additional details of floral scent characterization protocols in Adhikari et al., 2021).
Data Analysis
To assess phenotypic trait variation among A. cotula populations for plant height, plant volume, and dry biomass, a linear mixed model was used:
Where yijk is the response variable, αi is a fixed effect due to population, βj is a fixed effect due to block, (αβ)ij is a random effect of the population within the block, and ϵijk is the error term. The R package “lme4” was used for analysis (Bates et al., 2015). Residuals were examined for homogeneity of variance, and qq-plots were used to check for normality. For dependent variables that were counts, a generalized linear mixed model with a Poisson distribution and log link function were used instead, following the model framework described above. The count variables were days to seedling emergence, days to first budding, days to anthesis, days to first and last flowering, flowering duration (days), number of branches, number of flower heads, and plant life (days). For generalized linear models, the residual plots were also examined for homogeneity of variance. We calculated un-pooled variance from the models to assess the trait variation among populations. Estimated marginal means and post-hoc tests were made using the R package “emmeans” (Lenth et al., 2019). For flower size (cm2), floral VOC diversity, and evenness, for which fewer plants were sampled (see above) simple linear models, and for the number of ray florets, and VOC abundance and richness, generalized linear models with a Poisson distribution and log link function were used.
To assess if the population trait means or variances differed among populations, we conducted a permutational multivariate analysis of variance (PERMANOVA) on a Bray-Curtis dissimilarity matrix (Bray and Curtis, 1957) of ten phenotypic traits [plant height, total number of branches, first budding date, first flowering date, anthesis, plant life, flowering period, last day of flowering, total number of flower heads, and plant dry biomass]. Due to their different units and scales, all trait values were standardized by percent transformation using the function “decostand” in “vegan” package in R (Oksanen, 2019). For this analysis, only the traits measured on every plant in the study were selected, and so the traits such as floral scent VOCs, number of florets, and flower sizes were excluded (see above for the details). The package “vegan” was used for conducting PERMANOVA. Using the package “ggfortify,” principal component analysis biplots were created to show clusters of traits based on their similarity. Canonical correspondence analysis (CCA) was used to investigate whether climatic (mean annual precipitation, mean annual maximum and minimum temperatures, mean actual annual evapotranspiration, and mean annual water deficit) and edaphic (soil moisture) variables (Supplementary Table 1) across population origins were associated with any of the phenotypic traits. After calculating the Variance Inflation Factor (VIF) for each variable to test the goodness of fit in the CCA model, we removed all variables with >10 VIF (indicating strong collinearity with other variables and not contributing to variance explanation; Belsley et al., 2005), but kept mean annual minimum temperature and soil moisture for the final model. To determine whether the phenotypic trait correlations were associated with the geographical distances among populations, we tested the correlation between the Euclidean distance matrix of population sites and the Bray-Curtis dissimilarity matrix of phenotypic traits using a Mantel test (number of permutations = 999). The traits evaluated by the Mantel test were plant height, first flowering date, plant volume, total branches, last flowering, total flowers, first budding, flowering duration, anthesis, plant life, and dry mass. To show the clustering of different populations based on their phenotypic trait similarities, all trait values were standardized, and a dendrogram (agglomerative clustering method = “complete,” k = 4) was constructed. All data analyses were performed using R 3v.5.1 (R Development Core Team, 2018).
Results
Variance components and mean estimates for each trait were different among the 19 populations (Table 1). Anthemis cotula seedling emergence differed among populations (Figure 2; Table 1; Supplementary Table 2). The emergence in PNW populations ranged from 2 to 49% within 30 days of planting, and the emergence of Indian populations ranged from 55 to 72% during the same period. Most populations reached a similar plant volume by their first flowering, although Indian populations had a smaller volume than Tensed and Thornton populations (Figure 2; Table 1; Supplementary Table 2). Similarly, plants from Kambitsch, Spillman Farm, and Indian 1 populations flowered for the most extended periods (about 3 months), while plants from Cook Farm, Colfax, and St. John flowered for the shortest period (about 2 months) (Figure 2; Table 1; Supplementary Table 2). A shorter flowering period was associated with fewer total flower heads in the Cook Farm population but not in the Colfax and St. John populations (Figure 2; Table 1; Supplementary Table 2). Plants from Kambitsch, Spillman Farm, and Thornton populations lived for the longest periods (about 5 months) (Figure 2; Table 1; Supplementary Table 2), while plants from Cook Farm lived for <4 months. The total number of branches at first flowering also differed among populations. The Palouse population produced the greatest number of branches per plant, other populations producing intermediate numbers, and Spillman Farm and Thornton populations producing the fewest branches (Figure 2; Table 1; Supplementary Table 2). Individuals from Potlatch also had more branches than plants from the Spillman and Thornton populations. Tensed individuals had the greatest dry biomass at harvest, significantly greater than Indian, Spillman, and Genesee populations (Figure 2; Table 1; Supplementary Table 2). Anthesis and plant height at first flowering did not differ among populations (Figure 2; Table 1; Supplementary Table 2).
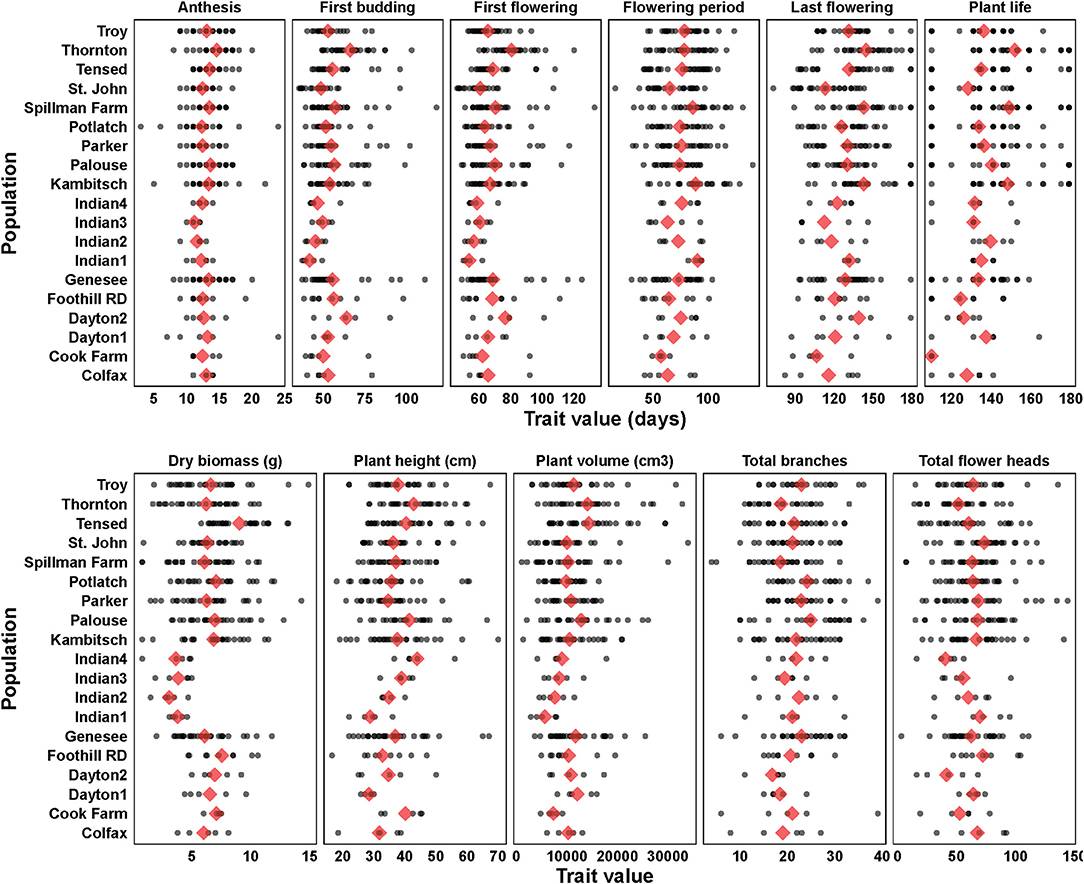
Figure 2. Phenotypic trait estimates (blue diamonds) and data points (black filled circles) among 19 A. cotula populations. Analyses were done with linear or generalized linear mixed effect models, where population and block were treated as fixed effects, and block and population interaction were treated as random effects. The mean estimates are from the post-hoc tests using emmeans (estimated marginal means or least-square means) in R.
The number of ray florets did not differ (F(18,76) = 0.36; p = 0.99) among populations, although two Indian populations tended to have fewer ray florets (mean ± SE: Indian population 2: 12.0 ± 1.6; Indian population 3: 12.8 ± 1.6), while Palouse (15.4 ± 1.8) and Cook Farm (15.8 ± 1.8) had the most. Flower size was marginally different (F(18,76) = 1.65; p = 0.07) among populations, and plants from Troy (2.25 ± 0.15 cm) had the smallest flowers while from St. John (3 ± 0.15 cm) had the largest flowers.
Floral scent differed among the tested populations (Table 2; F(10,78) = 3583262; p < 0.0001) with Dayton1 flowers producing the highest and Colfax the lowest total VOC concentration. Floral VOC richness also differed among populations, with the most compounds detected from Kambitsch (14.6 ± 1.3 compounds) and Palouse (14.6 ± 1.2) populations and the fewest from Colfax (6.5 ± 1.3) populations, while other populations were intermediate and similar to one another [Table 2; F(10,78) = 3.69; p < 0.001]. Floral VOCs diversity and evenness were also marginally different among tested populations [Table 2; diversity: F(10,78) = 1.72, p = 0.09 and evenness: F(10,78) = 1.93, p = 0.05)]. No VOCs variable was correlated with any of the edaphic or climatic variables (Supplementary Figure 2).
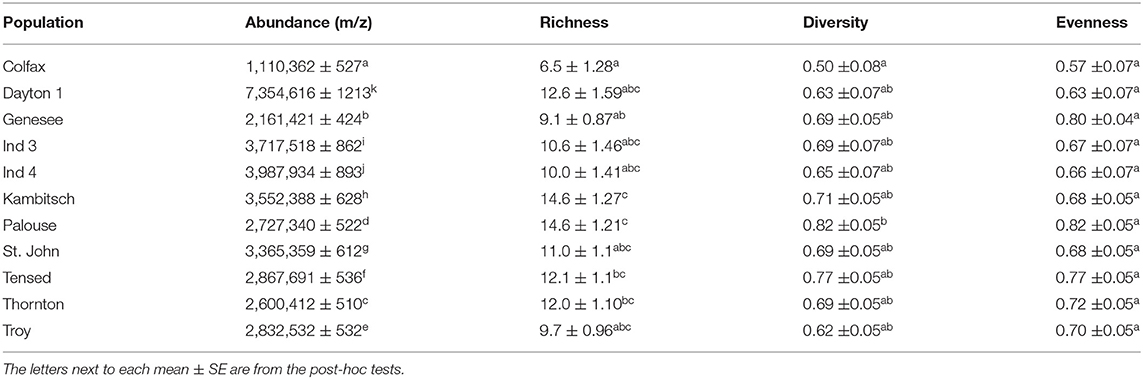
Table 2. Inter-population estimates (mean ± SE) among 11 populations in VOCs abundance, richness, diversity, and evenness collected per five flowers for 1 h.
PERMANOVA on a Bray-Curtis dissimilarity matrix of ten phenotypic traits [plant height, the total number of branches, first budding date, first flowering date, anthesis, plant life, flowering period, last day of flowering, the total number of flower heads, and plant dry biomass] indicated that trait composition among 19 populations was dissimilar (Figure 3; Pseudo-F(18, 307) = 3.18; r2 = 0.16; P = 0.001). The principal component biplot indicated that the first and second components combined explained 78.5% of the total variation (Figure 3). The total number of flower heads, flowering duration, first and last days of flowering, and plant life span contributed most to the observed dissimilarity in phenotypic trait composition among populations (Figure 3). CCA models showed that both climatic and edaphic variables did not explain much of the variation among phenotypic traits. Soil moisture (0.62%; Pseudo-F(1,302) = 1.9; P = 0.13), mean annual minimum temperature (0.30%; Pseudo-F(1,302) = 0.9; P = 0.42), and their interaction (0.35%; Pseudo-F(1,302) = 1.1; P = 0.35) combinedly explained only about 1.3% of the total variation in phenotypic traits, leaving a significant majority of variance proportion unexplained (Supplementary Figure 3).
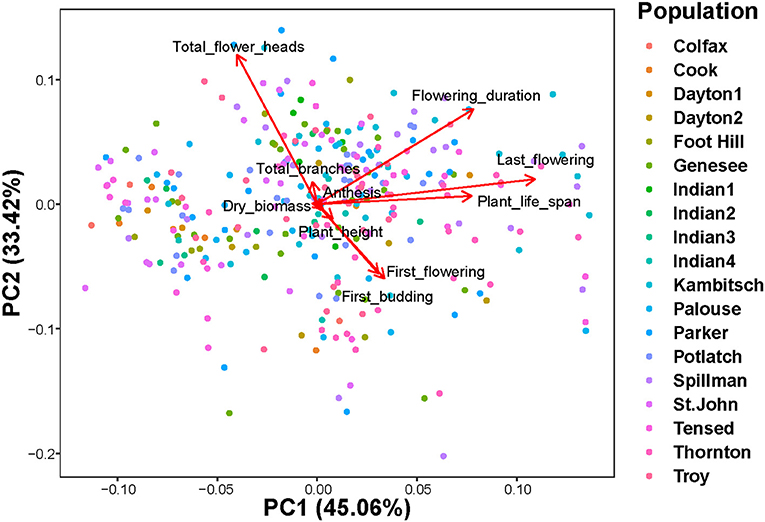
Figure 3. Principal component analysis biplot of ten selected phenotypic traits [plant height (cm), the total number of branches, first budding date (days), first flowering date (days), anthesis (days), plant life (days), flowering period (days), last day of flowering (days), the total number of flower heads, and plant dry biomass (g)] among 19 populations (PERMANOVA: Pseudo-F(18,307) = 3.18; r2 = 0.16; P = 0.001).
Most of the phenotypic traits were not strongly correlated with each other or with latitude, longitude, soil moisture or mean annual minimum temperature (Supplementary Figure 4). The total number of flower heads was negatively correlated with the initial date of budding or flowering but positively correlated with flowering duration and plant life span (Supplementary Figure 4). Our study indicated that geographical distance between A. cotula populations was not correlated to the similarity of phenotypic traits (Mantel test: r = 0.02, N = 306, p = 0.17, number of permutations = 999). Additionally, dendrogram clustering of 19 A. cotula populations based on the Bray-Curtis similarity of selected phenotypic traits indicated that populations did not cluster based on their geographical proximities (Supplementary Figure 5; Agglomerative coefficient = 0.66, method = “average,” k = 4).
Discussion
Trait Variation Among PNW Populations and Governing Processes
Our common garden study found a relatively high inter-population variation in phenotypic traits such as flowering period, the total number of flower heads, first budding, first and last flowering, plant life span, and floral scent VOCs among A. cotula populations. However, the trait variation was not associated with geographical distance. We present several processes that may explain inter-population trait variation among A. cotula populations and the potential implications for weed management.
Previous studies have reported that phenotypic traits of invasive taxa are associated with plants' invasiveness (Bossdorf et al., 2005; Pyšek and Richardson, 2008), but these traits have been measured at the species level, rarely among populations within a species of globally invasive annual crop weed. Knowledge of plant functional traits among locally adapted populations can inform invasive species management and forecast their distributions in the context of global environmental changes (Ehrlén and Morris, 2015; Peterson et al., 2019). The extent of trait variation in invading populations can be determined by local selection pressures, demographic history, genetic drift, and founder effects (Dlugosch and Parker, 2008; Hodgins and Rieseberg, 2011). Six non-exclusive processes alone or in combination potentially account for the trait variations among A. cotula populations in our study: selection by local environmental conditions and farming practices, human-aided or other natural movement of seeds across the landscape, trait-based clustering (i.e., population groupings based on their phenotypic traits) among geographically distant populations, and possible genetic drift.
Evidence for each of these processes differed. First, we found weak and variable patterns of local adaptation in A. cotula populations. In general, the plants in drier sites (Supplementary Table 1) flowered for a shorter period yet produced a similar number of flower heads compared to those of wetter sites. For example, plants from Kambitsch and Spillman (Supplementary Table 1; mean annual precipitation: 625 and 545 mm, respectively) flowered for ~1 month longer than plants from Cook farm, Colfax, and St. John (mean annual precipitation: 625 mm, 508 mm, and 457 mm, respectively). The shorter flowering periods were associated with reduced flower head production in plants from Cook Farm, but not in Colfax and St. John, suggesting that plants in drier regions tend to maximize the flower production within the compressed flowering season (Franks et al., 2007; Shavrukov et al., 2017). Also, Troy plants (mean annual precipitation: 617 mm) had the smallest flowers, while St. John (457 mm) had the largest flowers, consistent with greater investment in flowers that could compensate for a shorter season and exposure to pollinators. Alternatively, as it occurs with some other invasive plant species (e.g., Miller et al., 2018), the long flowering period in some A. cotula populations might help compete for pollinators that they share with native species. Variation in floral scent VOCs among A. cotula populations also could reflect adaptation to local environmental conditions such as soil moisture (Burkle and Runyon, 2016; Campbell et al., 2019) and the diversity and abundance of local pools of flower visitors (Burkle and Runyon, 2019; Kantsa et al., 2019). Although these patterns in our data could result from local adaptation, climatic and edaphic variables explained only a small proportion (0.013, i.e., 1.3%) of the total variation in phenotypic traits.
Second, A. cotula in the PNW mainly occurs in agricultural fields, hence localized differences in agricultural management practices such as the competitiveness of the planted crop (Ogg et al., 1994), crop rotations, herbicide applications (Lyon et al., 2017, Hovick et al., 2018), tillage (Ghersa and Martínez-Ghersa, 2000), and fertilizer application (Zeng et al., 2017) could have selected for local adaptation in A. cotula traits across populations. For example, farming practices including tillage, fertilizer, herbicide regime, crop type, sowing date, and fallow can affect the flowering phenology of associated weeds (Ekeleme et al., 2000; Fried et al., 2012; Gaba et al., 2017). Unfortunately, we were unable to collect precise details of farming history from all seed collecting sites, so whether farming practices affect A. cotula in our study populations cannot be evaluated.
Third, trait variation could reflect human-aided movement of seed, among geographically unrelated A. cotula populations, as occurs for other weeds (Shimono et al., 2010, 2020), on farming equipment, and with farming inputs (Blanco-Moreno et al., 2004). Several human-mediated seed contamination pathways can occur during crop production, harvesting, and crop handling (Wilson et al., 2016; Gao et al., 2018). The trait-based clustering of A. cotula populations in our study was not associated with geographical proximity, as expected from landscape-scale geneflow, but was geospatially random. For example, based on the similarities of traits such as anthesis, flowering period, last day of flowering, plant life, branches, dry biomass, and total flower heads, plants from Potlatch and Palouse were clustered together, but the Palouse site is geographically farther from Potlatch than from many other sites (Figure 1, Supplementary Figure 5, Supplementary Table 1). The human-aided movement of seeds between Potlatch and Palouse might have caused these sites' similarity and other clustering among sites in the region. Again, records of equipment movements among sites or via common routes in the region are not available to decipher this mechanism.
Fourth, A. cotula was first reported more than 143 years ago from the PNW (Adhikari et al., 2020a), and the species could have been independently introduced multiple times in the region. The original variation of the introduced populations, genetic drift, and the number and frequency of introduction events in the past are unknown, but each A. cotula population in the PNW could be a composite of multiple genetically unique seed sources. The agricultural practices or variable climate that favors different traits in different years could have maintained the genetic diversity in the initial infestation(s).
Fifth, the randomly introduced small populations isolated geographically after their introduction (s) might have undergone genetic drift. Genetic drift can be a strong evolutionary force in determining traits' development (Keller and Taylor, 2008; Luo et al., 2015; Boaventura-Novaes et al., 2018; Banerjee et al., 2019) and increasing genetic and phenotypic differences between populations (Willi et al., 2007). Hence, it is possible that A. cotula populations could have received different origins and have independently experienced genetic drift in the past, causing trait variation.
Thus, none of the potential drivers of interpopulation A. cotula trait variation can be ruled out, and it is possible that several or all contributed to varying degrees. If these factors are operating simultaneously, they may also interact in their effects on the weed. Variable farm management practices such as the timing of planting or other mechanical weed management inputs in crop fields (Hovick et al., 2018) will be influenced by climatic and edaphic factors such that both climate and farming practice together exert unique selection on specific populations. Variations among populations could reflect long and differing histories of selection, drift, or pre-adaptation in the native range before introduction (Keller and Taylor, 2008; Schlaepfer et al., 2010; Cadotte et al., 2018).
Indian Populations
The Indian populations included in this study and the PNW populations allow the detection of other potentially important patterns in A. cotula traits. Most of the traits including plant height, the total number of branches, first budding date, first flowering date, anthesis, plant life, flowering period, last day of flowering, flower size, and the number of florets in Indian populations were mostly similar with PNW populations, potentially suggesting that they are genetically similar with at least some PNW populations. However, Indian populations emerged earlier, emitted fewer VOCs, and produced smaller plant biomass and fewer flower heads than most PNW populations. Under our experiment's conditions, Indian populations consistently had greater seedling emergence rates than the 15 PNW populations. The difference in seedling emergence could reflect differences in age of seed or conditions of seed collection and storage between Indian and PNW populations. Indian seeds were collected in 2017, while those from PNW1 populations were collected in 2018. Previous studies have shown that A. cotula germination is lower in the first year than the second and third year after production (Roberts and Neilson, 1981). On the other hand, the seedling emergence of Indian populations was also greater than PNW2 populations which were collected in 2017 or earlier. If an environmental artifact can be ruled out, the differences between Indian and PNW populations could reflect differences in selective regimes invasion histories of A. cotula on the Indian and PNW collection sites.
Germinability is a crucial reproductive fitness trait. Species with greater germinability over wider climatic and edaphic conditions have greater potential invasiveness (Pyšek and Richardson, 2008). The Indian populations were from lower latitude and higher elevation than the PNW sites (Supplementary Table 1, Supplementary Figure 1) and from uncultivated habitats with shorter introduction history (ca. 50 years) rather than cultivated agricultural fields with more extended introduction history (ca.143 years) as in the PNW. Despite their similar (Mediterranean-like) seasonality, the climate regimes of the Indian sites are wetter and warmer than those of PNW sites (Supplementary Table 1). These differences may have selected for a different emergence rate, at least in the relatively warm and well-watered conditions of the greenhouse used in this study. Previous studies have reported that population origins' latitude and local environmental conditions could affect germination rates of seeds (Jonas and Geber, 1999; Gaba et al., 2017). Early emergence, in general, is typical of invasive species, particularly in semi-arid ecosystems (Funk, 2013; Gioria and Pyšek, 2017), but as shown in the previous studies (Schutte et al., 2012; Davis et al., 2013; Hovick et al., 2018), natural (i.e., Indian) populations in our research emerged earlier than those of agricultural (i.e., PNW) populations.
Despite revealing some interesting information on phenotypic trait variation among A. cotula populations, our study was limited by not having samples from the native range of A. cotula. While we have included A. cotula populations from its two key invading ranges (PNW, USA and Kashmir Valley, India) with Mediterranean-like climates, we were unable to obtain seeds from the native range (Mediterranean region) which would have allowed us to compare the invasive populations with those of native ones. Even though we included 15 traits across four phenotypic categories (morphological, phenological, reproductive, and physiological), we were unable to assess other traits (e.g., leaf number and specific leaf area, seed dormancy, stress tolerance etc.) that could also be associated with the invasiveness of A. cotula. Also, although A. cotula is globally distributed (Adhikari et al., 2020a), our study represented only two major continents: North America and Asia. Nevertheless, our study has provided an important baseline information on invasive A. cotula traits, and the future studies are required to investigate more traits of neophytes (i.e., introduced after the Columbian Exchange), archaeophytes, and native populations.
Implications for Weed Management in PNW and Other Agroecosystems
Whether the trait variation observed among A. cotula populations resulted from local adaptation to climatic conditions, farming practices, human-aided movements of seeds, multiple introductions of genetically unique seed sources, genetic drift, or some combination of these drivers, the variation could facilitate A. cotula invasiveness. Trait variation enables the existence of invasive populations under various competitive abiotic and biotic conditions (Violle et al., 2012; Lemke et al., 2015). If the variation in phenotypic traits were a result of local adaptation, then populations could persist in a wide range of habitats (Sultan, 1995; Thompson and Fronhofer, 2019), potentially complicating weed management in highly variable agricultural landscapes (Clements et al., 2004; Hovick et al., 2018). For example, the adaptation of early and shorter flowering periods of populations in the drier sites dictates a different window for herbicide treatments to prevent reseeding than those in the wetter areas. Also, population-specific differences in emergence time (i.e., early vs. late) may require site-specific weed control practices. As A. cotula is an obligate out-crosser and a generalist species (Kay, 1971; Adhikari et al., 2020a), plasticity, as expressed by floral scent profiles, could be critical to attract a wide range of pollinators across sites, which could promote invasiveness by ensuring high seed set and even competing with native species for pollination services (Montero-Castaño et al., 2014; David et al., 2017). Hence, the weed management plan for A. cotula in agricultural fields of the PNW may need to be tailored to specific locations instead of a uniformly adopted plan across the region (Sterling et al., 2004). The management plan should also consider that trait variation is an ongoing process in response to climatic, edaphic, and management regimes that potentially facilitate accumulated advantages and promote weed invasiveness, becoming more challenging to manage over time.
The human-aided random movement of seeds across various sites could also complicate weed management plans. For example, human-aided herbicide-resistant seed movement among sites or introduction to the new site where frequent human movement (e.g., with direct road connections and trade routes) occurs would require similar management practices such as applying herbicides of similar modes of action than to the sites with less frequent human movement and with no herbicide-resistant populations. Some A. cotula populations in our study differ in herbicide resistance (Adhikari, Burke, Eigenbrode, unpublished), making the weed more serious as practices change while the new herbicide chemistries may not be available as quickly. The trait variation among the A. cotula population could impede weed management in different climatic zones, so understanding the biology, variability, and whether the weed is currently under selection for management-important traits across the region will help implement optimal management during the current transition of adopting climate-change-resilient diversified cropping systems.
Data Availability Statement
The raw data supporting the conclusions of this article will be made available by the authors, without undue reservation.
Author Contributions
SA, ICB, and SDE designed the study. ICB and SDE acquired the funding and supervised the study. SA contributed to the seed collection, greenhouse experiment, data analysis, and manuscript preparation. JP contributed to data analysis. All authors contributed to the article, edited, and approved the submitted version.
Funding
This research was part of the LIT (Landscapes in Transition) project, supported by award #2017-68002-26819 and Hatch project 1017286 from the USDA National Institute of Food and Agriculture.
Conflict of Interest
The authors declare that the research was conducted in the absence of any commercial or financial relationships that could be construed as a potential conflict of interest.
Acknowledgments
We thank Dr. Manzoor Shah (University of Kashmir, India), scientists from the eastern US, and PNW producers for providing A. cotula seeds. We thank Philip Anderson for greenhouse space and support, Grace Overlie for seed counting, and Vincent Oliveras for data collection assistance.
Supplementary Material
The Supplementary Material for this article can be found online at: https://www.frontiersin.org/articles/10.3389/fagro.2021.662375/full#supplementary-material
References
Adhikari, A., Rew, L. J., Mainali, K. P., Adhikari, S., and Maxwell, B. D. (2020b). Future distribution of invasive weed species across the major road network in the state of Montana, USA. Reg. Environ. Chang. 20, 1–14. doi: 10.1007/s10113-020-01671-0
Adhikari, S., Adhikari, A., Weaver, D. K., Bekkerman, A., and Menalled, F. D. (2019). Impacts of agricultural management systems on biodiversity and ecosystem services in highly simplified dryland landscapes. Sustainability 11:3223. doi: 10.3390/su11113223
Adhikari, S., Burke, I. C., Revolinski, S. R., Piaskowski, J., and Eigenbrode, S. D. (2021). Within-population trait variation in a globally invasive plant species Mayweed chamomile (Anthemis cotula): implications for future invasion and management. Front. Agron. 3:640208. doi: 10.3389/fagro.2021.640208
Adhikari, S., Burke, I. C., and Eigenbrode, S. D. (2020a). Mayweed chamomile (Anthemis cotula L.) biology and management—a review of an emerging global invader. Weed Res. 60, 313–322. doi: 10.1111/wre.12426
Bai, W. N., Wang, W. T., and Zhang, D. Y. (2014). Contrasts between the phylogeographic patterns of chloroplast and nuclear DNA highlight a role for pollen-mediated gene flow in preventing population divergence in an East Asian temperate tree. Mol. Phylogenet. Evol. 81, 37–48. doi: 10.1016/j.ympev.2014.08.024
Banerjee, A. K., Guo, W., and Huang, Y. (2019). Genetic and epigenetic regulation of phenotypic variation in invasive plants - linking research trends towards a unified framework. NeoBiota 49, 77–103. doi: 10.3897/neobiota.49.33723
Bates, D., Mächler, M., Bolker, B. M., and Walker, S. C. (2015). Fitting linear mixed-effects models using lme4. J. Stat. Softw. 67, 1–48. doi: 10.18637/jss.v067.i01
Baythavong, B. S. (2011). Linking the spatial scale of environmental variation and the evolution of phenotypic plasticity: selection favors adaptive plasticity in fine-grained environments. Am. Nat. 178, 75–87. doi: 10.1086/660281
Belsley, D. A., Kuh, E., and Welsch, R. E. (2005). Regression Diagnostics: Identifying Influential Data and Sources of Collnearity. New York, NY: John Wiley & Sons, Inc. p. 292.
Blanco-Moreno, J. M., Chamorro, L., Masalles, R. M., Recasens, J., and Sans, F. X. (2004). Spatial distribution of Lolium rigidum seedlings following seed dispersal by combine harvesters. Weed Res. 44, 375–387. doi: 10.1111/j.1365-3180.2004.00412.x
Boaventura-Novaes, C. R. D., Novaes, E., Mota, E. E. S., Telles, M. P. C., Coelho, A. S. G., and Chaves, L. J. (2018). Genetic drift and uniform selection shape evolution of most traits in Eugenia dysenterica DC. (Myrtaceae). Tree Genet. Genomes 14, 1–14. doi: 10.1007/s11295-018-1289-2
Bossdorf, O., Auge, H., Lafuma, L., Rogers, W. E., Siemann, E., and Prati, D. (2005). Phenotypic and genetic differentiation between native and introduced plant populations. Oecologia 144, 1–11. doi: 10.1007/s00442-005-0070-z
Bradley, B. A., Blumenthal, D. M., Wilcove, D. S., and Ziska, L. H. (2010). Predicting plant invasions in an era of global change. Trends Ecol. Evol. 25, 310–318. doi: 10.1016/j.tree.2009.12.003
Bray, J. R., and Curtis, J. T. (1957). An ordination of the upland forest communities of southern Wisconsin. Ecol. Monogr. 27, 325–349. doi: 10.2307/1942268
Burkle, L. A., and Runyon, J. B. (2016). Drought and leaf herbivory influence floral volatiles and pollinator attraction. Glob. Chang. Biol. 22, 1644–1654. doi: 10.1111/gcb.13149
Burkle, L. A., and Runyon, J. B. (2019). Floral volatiles structure plant–pollinator interactions in a diverse community across the growing season. Funct. Ecol. 33, 1365–2435. doi: 10.1111/1365-2435.13424
CABI (2018). Anthemis cotula (dog fennel). Available online at: https://www.cabi.org/isc/datasheet/5672 (accessed January 5, 2020).
Cadotte, M. W., Campbell, S. E., Li, S., Sodhi, D. S., and Mandrak, N. E. (2018). Preadaptation and naturalization of nonnative species: darwin's two fundamental insights into species invasion. Annu. Rev. Plant Biol. 69, 661–684. doi: 10.1146/annurev-arplant-042817-040339
Campbell, D. R., Sosenski, P., and Raguso, R. A. (2019). Phenotypic plasticity of floral volatiles in response to increasing drought stress. Ann. Bot. 123, 601–610. doi: 10.1093/aob/mcy193
Chaudhary, A., Pfister, S., and Hellweg, S. (2016). Spatially explicit analysis of biodiversity loss due to global agriculture, pasture and forest land use from a producer and consumer perspective. Environ. Sci. Technol. 50, 3928–3936. doi: 10.1021/acs.est.5b06153
Chongtham, S. K., Devi, L., Singh, R. P., Jat, A. L., Lhungdim, J., Bhupenchandra, I., et al. (2019). Crop-weed interactions and their management under climate change: a review. Int. J. Chem. Stud. 7, 4498–4505. Available online at: https://www.chemijournal.com/archives/2019/vol7issue3/PartBV/7-3-421-304.pdf
Clements, D. R., Ditommaso, A., Jordan, N., Booth, B. D., Cardina, J., Doohan, D., et al. (2004). Adaptability of plants invading North American cropland. Agric. Ecosyst. Environ. 104, 379–398. doi: 10.1016/j.agee.2004.03.003
Darlington, H. T. (1931). The 50-year period for Dr. Beal's seed viability experiment. Am. J. Bot. 18:262. doi: 10.2307/2435902
David, P., Thébault, E., Anneville, O., Duyck, P. F., Chapuis, E., and Loeuille, N. (2017). “Impacts of invasive species on food webs: a review of empirical data,” in Advances in Ecological Research (Cambridge, MA: Academic Press Inc.), 1–60. doi: 10.1016/bs.aecr.2016.10.001
Davis, A. S., Clay, S., Cardina, J., Dille, A., Forcella, F., Lindquist, J., et al. (2013). Seed burial physical environment explains departures from regional hydrothermal model of giant ragweed (Ambrosia trifida) seedling emergence in U.S. Midwest. Weed Sci. 61, 415–421. doi: 10.1614/WS-D-12-00139.1
Dlugosch, K. M., and Parker, I. M. (2008). Founding events in species invasions: genetic variation, adaptive evolution, and the role of multiple introductions. Mol. Ecol. 17, 431–449. doi: 10.1111/j.1365-294X.2007.03538.x
Douglas, C. L., R. W Rickman, B. L., Klepper, J. F., and Wysocki, Z. D. J. (1992). Agroclimatic zones for dryland winter wheat producing areas of Idaho, Washington, and Oregon. Available online at: https://research.wsulibs.wsu.edu/xmlui/handle/2376/1603 (accessed December 19, 2019).
Ehrlén, J., and Morris, W. F. (2015). Predicting changes in the distribution and abundance of species under environmental change. Ecol. Lett. 18, 303–314. doi: 10.1111/ele.12410
Eigenbrode, S. D., Capalbo, S. M., Houston, L. L., Johnson-Maynard, J., Kruger, C., and Olen, B. (2013). “Agriculture: impacts, adaptation, and mitigation,” in Climate Change in the Northwest: Implications for Our Landscapes, Waters, and Communities (Washington, DC: Island Press-Center for Resource Economics), 149–180. doi: 10.5822/978-1-61091-512-0_6
Ekeleme, F., Akobundu, I. O., Isichei, A. O., and Chikoye, D. (2000). Influence of fallow type and land-use intensity on weed seed rain in a forest/savanna transition zone. Weed Sci. 48, 604–612. doi: 10.1614/0043-1745(2000)048[0604:IOFTAL]2.0.CO;2
Franks, S. J., Sim, S., and Weis, A. E. (2007). Rapid evolution of flowering time by an annual plant in response to a climate fluctuation. Proc. Natl. Acad. Sci. U.S.A. 104, 1278–1282. doi: 10.1073/pnas.0608379104
Fried, G., Kazakou, E., and Gaba, S. (2012). Trajectories of weed communities explained by traits associated with species' response to management practices. Agric. Ecosyst. Environ. 158, 147–155. doi: 10.1016/j.agee.2012.06.005
Funk, J. L. (2013). The physiology of invasive plants in low-resource environments. Conserv. Physiol. 1, 1–17. doi: 10.1093/conphys/cot026
Gaba, S., Perronne, R., Fried, G., Gardarin, A., Bretagnolle, F., Biju-Duval, L., et al. (2017). Response and effect traits of arable weeds in agro-ecosystems: a review of current knowledge. Weed Res. 57, 123–147. doi: 10.1111/wre.12245
Gao, P., Zhang, Z., Sun, G., Yu, H., and Qiang, S. (2018). The within-field and between-field dispersal of weedy rice by combine harvesters. Agron. Sustain. Dev. 38, 1–10. doi: 10.1007/s13593-018-0518-2
Ghersa, C. M., and Martínez-Ghersa, M. A. (2000). Ecological correlates of weed seed size and persistence in the soil under different tilling systems: implications for weed management. F. Crop. Res. 67, 141–148. doi: 10.1016/S0378-4290(00)00089-7
Gioria, M., and Pyšek, P. (2017). Early bird catches the worm: germination as a critical step in plant invasion. Biol. Invasions 19, 1055–1080. doi: 10.1007/s10530-016-1349-1
Global Biodiversity Information Facility. (2018). Occurrence Status. Available online at: https://www.gbif.org/occurrence/search?q=Anthemis%20cotula (accessed November 05, 2018).
Hodgins, K. A., and Rieseberg, L. (2011). Genetic differentiation in life-history traits of introduced and native common ragweed (Ambrosia artemisiifolia) populations. J. Evol. Biol. 24, 2731–2749. doi: 10.1111/j.1420-9101.2011.02404.x
Hokanson, S. C., Grumet, R., and Hancock, J. F. (1997). Effect of border rows and trap/donor ratios on pollen-mediated gene movement. Ecol. Appl. 7, 1075–1081. doi: 10.1890/1051-0761(1997)007[1075:EOBRAT]2.0.CO;2
Hovick, S. M., McArdle, A., Harrison, S. K., and Regnier, E. E. (2018). A mosaic of phenotypic variation in giant ragweed (Ambrosia trifida): local- and continental-scale patterns in a range-expanding agricultural weed. Evol. Appl. 11, 995–1009. doi: 10.1111/eva.12614
Ilnicki, R., and Johnson, M. W. (1959). “Temperature, light and seed size and their,” in Proceedings of 13th Northeast Weed Control Conference (King Ferry, NY: Northeastern Weed Science Society), 440–440.
Jonas, C. S., and Geber, M. A. (1999). Variation among populations of Clarkia Unguiculata (Onagraceae) along altitudinal and latitudinal gradients. Am. J. Bot. 86, 333–343. doi: 10.2307/2656755
Joshi, J., Schmid, B., Caldeira, M. C., Dimitrakopoulos, P. G., Good, J., Harris, R., et al. (2001). Local adaptation enhances performance of common plant species. Ecol. Lett. 4, 536–544. doi: 10.1046/j.1461-0248.2001.00262.x
Kantsa, A., Raguso, R. A., Lekkas, T., Kalantzi, O. I., and Petanidou, T. (2019). Floral volatiles and visitors: a meta-network of associations in a natural community. J. Ecol. 107, 2574–2586. doi: 10.1111/1365-2745.13197
Karimi, T., Stöckle, C. O., Higgins, S., and Nelson, R. (2018). Climate change and dryland wheat systems in the US Pacific Northwest. Agric. Syst. 159, 144–156. doi: 10.1016/j.agsy.2017.03.014
Keller, S. R., and Taylor, D. R. (2008). History, chance and adaptation during biological invasion: separating stochastic phenotypic evolution from response to selection. Ecol. Lett. 11, 852–866. doi: 10.1111/j.1461-0248.2008.01188.x
Lemke, I. H., Kolb, A., Graae, B. J., De Frenne, P., Acharya, K. P., Blandino, C., et al. (2015). Patterns of phenotypic trait variation in two temperate forest herbs along a broad climatic gradient. Plant Ecol. 216, 1523–1536. doi: 10.1007/s11258-015-0534-0
Lenth, R. V., Buerkner, P., Herve, M., Love, J., Riebl, H., and Singmann, H. (2019). R Package “emmeans”.Estimated Marginal Means, aka Least-Squares Means. Available online at: https://cran.r-project.org/web/packages/emmeans/index.html
Luo, Y., Widmer, A., and Karrenberg, S. (2015). The roles of genetic drift and natural selection in quantitative trait divergence along an altitudinal gradient in Arabidopsis thaliana. Heredity (Edinb). 114, 220–228. doi: 10.1038/hdy.2014.89
Lyon, D. J., Burke, I. C., Hulting, A. G., and Campbell, J. M. (2017). Integrated Management of Mayweed Chamomile in Wheat and Pulse Crop Production Systems. Washington State University Extension Publications PNW695. Pullman, WA: Washington State University, 1–6.
Mack, R. N., and Erneberg, M. (2002). The United States naturalized flora: largely the product of deliberate introductions. Ann. Missouri Bot. Gard. 89:176. doi: 10.2307/3298562
Majetic, C. J., Raguso, R. A., and Ashman, T.-L. (2009). The sweet smell of success: floral scent affects pollinator attraction and seed fitness in Hesperis matronalis. Funct. Ecol. 23, 480–487. doi: 10.1111/j.1365-2435.2008.01517.x
Matzrafi, M., Brunharo, C., Tehranchian, P., Hanson, B. D., and Jasieniuk, M. (2019). Increased temperatures and elevated CO2 levels reduce the sensitivity of Conyza canadensis and Chenopodium album to glyphosate. Sci. Rep. 9, 1–11. doi: 10.1038/s41598-019-38729-x
Miller, C. M., Barratt, B. I. P., Dickinson, K. J. M., and Lord, J. M. (2018). Are introduced plants a threat to native pollinator services in montane–alpine environments? Alp. Bot. 128, 179–189. doi: 10.1007/s00035-018-0206-5
Montero-Castaño, A., Vilà, M., and Ortiz-Sánchez, F. J. (2014). Pollination ecology of a plant in its native and introduced areas. Acta Oecol. 56, 1–9. doi: 10.1016/j.actao.2014.01.001
Oerke, E. C. (2006). Crop losses to pests. J. Agric. Sci. 144, 31–43. doi: 10.1017/S0021859605005708
Ogg, A. G., Stephens, R. H., and Gealy, D. R. (1994). Interference between Mayweed chamomile (Anthemis cotula) and pea (Pisum sativum) is affected by form of interference and soil water regime. Weed Sci. 42, 579–585. doi: 10.1017/S0043174500076980
Ohadi, S., Hodnett, G., Rooney, W., and Bagavathiannan, M. (2017). Gene flow and its consequences in Sorghum spp. CRC. Crit. Rev. Plant Sci. 36, 367–385. doi: 10.1080/07352689.2018.1446813
Oksanen, J. (2019). CRAN - Package vegan. Available online at: https://cran.r-project.org/web/packages/vegan/index.html (accessed January 10, 2020).
O'Leary, G., Aggarwal, P., Calderini, D., Connor, D., Craufurd, P., Eigenbrode, S., et al. (2018). Challenges and responses to ongoing and projected climate change for dryland cereal production systems throughout the World. Agronomy 8:34. doi: 10.3390/agronomy8040034
Peterson, M. L., Doak, D. F., and Morris, W. F. (2019). Incorporating local adaptation into forecasts of species' distribution and abundance under climate change. Glob. Chang. Biol. 25, 775–793. doi: 10.1111/gcb.14562
Pielou, E. C. (1966). Species-diversity and pattern-diversity in the study of ecological succession. J. Theor. Biol. 10, 370–383. doi: 10.1016/0022-5193(66)90133-0
Pimentel, D., McNair, S., Janecka, J., Wightman, J., Simmonds, C., O'Connell, C., et al. (2001). Economic and environmental threats of alien plant, animal, and microbe invasions. Agric. Ecosyst. Environ. 84, 1–20. doi: 10.1016/S0167-8809(00)00178-X
Pimentel, D., Zuniga, R., and Morrison, D. (2005). Update on the environmental and economic costs associated with alien-invasive species in the United States. Ecol. Econ. 52, 273–288. doi: 10.1016/j.ecolecon.2004.10.002
Pratt, C. F., Constantine, K. L., and Murphy, S. T. (2017). Economic impacts of invasive alien species on African smallholder livelihoods. Glob. Food Sec. 14, 31–37. doi: 10.1016/j.gfs.2017.01.011
Pyšek, P., and Richardson, D. M. (2008). “Traits associated with invasiveness in alien plants: where do we stand?,” in Biological Invasions (Berlin: Springer Berlin Heidelberg), 97–125. doi: 10.1007/978-3-540-36920-2_7
Pyšek, P., Hulme, P. E., Simberloff, D., Bacher, S., Blackburn, T. M., Carlton, J. T., et al. (2020). Scientists' warning on invasive alien species. Biol. Rev. 95, 1511–1534. doi: 10.1111/brv.12627
R Development Core Team (2018). R: A language and environment for statistical computing. Available online at: https://www.r-project.org/ (accessed June 8, 2020).
Richards, C. L., Bossdorf, O., Muth, N. Z., Gurevitch, J., and Pigliucci, M. (2006). Jack of all trades, master of some? On the role of phenotypic plasticity in plant invasions. Ecol. Lett. 9, 981–993. doi: 10.1111/j.1461-0248.2006.00950.x
Roberts, H. A., and Neilson, J. E. (1981). Seed survival and periodicity of seedling emergence in twelve weedy species of Compositae. Ann. Appl. Biol. 97, 325–334. doi: 10.1111/j.1744-7348.1981.tb05119.x
Rollin, O., Benelli, G., Benvenuti, S., Decourtye, A., Wratten, S. D., Canale, A., et al. (2016). Weed-insect pollinator networks as bio-indicators of ecological sustainability in agriculture. A review. Agron. Sustain. Dev. 36, 1–22. doi: 10.1007/s13593-015-0342-x
Rowe, L., Gibson, D., Bahlai, C. A., Gibbs, J., Landis, D. A., and Isaacs, R. (2020). Flower traits associated with the visitation patterns of bees. Oecologia. 193, 511–522. doi: 10.1007/s00442-020-04674-0
Schiestl, F. P. (2015). Ecology and evolution of floral volatile-mediated information transfer in plants. New Phytol. 206, 571–577. doi: 10.1111/nph.13243
Schlaepfer, D. R., Glättli, M., Fischer, M., and Van Kleunen, M. (2010). A multi-species experiment in their native range indicates pre-adaptation of invasive alien plant species. New Phytol. 185, 1087–1099. doi: 10.1111/j.1469-8137.2009.03114.x
Schutte, B. J., Regnier, E. E., and Harrison, S. K. (2012). Seed dormancy and adaptive seedling emergence timing in giant ragweed (Ambrosia trifida). Weed Sci. 60, 19–26. doi: 10.1614/WS-D-11-00049.1
Shah, M. A., Reshi, Z., and Rashid, I. (2008). Mycorrhizal source and neighbour identity differently influence Anthemis cotula L. invasion in the Kashmir Himalaya, India. Appl. Soil Ecol. 40, 330–337. doi: 10.1016/j.apsoil.2008.06.002
Shah, M. A., Reshi, Z. A., and Khasa, D. P. (2009). Arbuscular mycorrhizas: drivers or passengers of alien plant invasion. Bot. Rev. 75, 397–417. doi: 10.1007/s12229-009-9039-7
Sharma, S., Walia, S., Rathore, S., Kumar, P., and Kumar, R. (2020). Combined effect of elevated CO2 and temperature on growth, biomass and secondary metabolite of Hypericum perforatum L. in a western Himalayan region. J. Appl. Res. Med. Aromat. Plants 16:100239. doi: 10.1016/j.jarmap.2019.100239
Shavrukov, Y., Kurishbayev, A., Jatayev, S., Shvidchenko, V., Zotova, L., Koekemoer, F., et al. (2017). Early flowering as a drought escape mechanism in plants: how can it aid wheat production? Front. Plant Sci. 8, 1–8. doi: 10.3389/fpls.2017.01950
Shimono, A., Kanbe, H., Nakamura, S., Ueno, S., Yamashita, J., and Asai, M. (2020). Initial invasion of glyphosate-resistant Amaranthus palmeri around grain-import ports in Japan. Plants People Planet 2, 640–648. doi: 10.1002/ppp3.10156
Shimono, Y., and Konuma, A. (2008). Effects of human-mediated processes on weed species composition in internationally traded grain commodities. Weed Res. 48, 10–18. doi: 10.1111/j.1365-3180.2008.00605.x
Shimono, Y., Takiguchi, Y., and Konuma, A. (2010). Contamination of internationally traded wheat by herbicide-resistant Lolium rigidum. Weed Biol. Manag. 10, 219–228. doi: 10.1111/j.1445-6664.2010.00387.x
Smitchger, J. A., Burke, I. C., and Yenish, J. P. (2012). The critical period of weed control in lentil (Lens culinaris) in the Pacific Northwest. Weed Sci. 60, 81–85.
Sterling, T. M., Thompson, D. C., and Abbott, L. B. (2004). Implications of invasive plant variation for weed management 1. Weed Technol. 18, 1319–1324. doi: 10.1614/0890-037X(2004)018[1319:IOIPVF]2.0.CO;2
Stewart, R. R. (1972). An Annotated Catalogue of the Vascular Plants of West Pakistan and Kashmir. Karachi: Fakhri Print Press, 1028.
Sultan, S. E. (1995). Phenotypic plasticity and plant adaptation. Acta Botanica Neerlandica. 44, 363–383. doi: 10.1111/j.1438-8677.1995.tb00793.x
Thompson, P. L., and Fronhofer, E. A. (2019). The conflict between adaptation and dispersal for maintaining biodiversity in changing environments. bioRxiv, 490722. doi: 10.1073/pnas.1911796116
Tilman, D., Balzer, C., Hill, J., and Befort, B. L. (2011). Global food demand and the sustainable intensification of agriculture. Proc. Natl. Acad. Sci. U.S.A. 108, 20260–20264. doi: 10.1073/pnas.1116437108
Van Kleunen, M., Dawson, W., Essl, F., Pergl, J., Winter, M., Weber, E., et al. (2015). Global exchange and accumulation of non-native plants. Nature 525, 100–103. doi: 10.1038/nature14910
Vander Zanden, M. J., Hansen, G. J. A., Higgins, S. N., and Kornis, M. S. (2010). A pound of prevention, plus a pound of cure: early detection and eradication of invasive species in the Laurentian Great Lakes. J. Great Lakes Res. 36, 199–205. doi: 10.1016/j.jglr.2009.11.002
Vilà, M., Espinar, J. L., Hejda, M., Hulme, P. E., Jarošík, V., Maron, J. L., et al. (2011). Ecological impacts of invasive alien plants: a meta-analysis of their effects on species, communities and ecosystems. Ecol. Lett. 14, 702–708. doi: 10.1111/j.1461-0248.2011.01628.x
Violle, C., Enquist, B. J., McGill, B. J., Jiang, L., Albert, C. H., Hulshof, C., et al. (2012). The return of the variance: intraspecific variability in community ecology. Trends Ecol. Evol. 27, 244–252. doi: 10.1016/j.tree.2011.11.014
Weisberger, D., Nichols, V., and Liebman, M. (2019). Does diversifying crop rotations suppress weeds? A meta-analysis. PLoS ONE 14, 1–12. doi: 10.1371/journal.pone.0219847
Willi, Y., Van Buskirk, J., Schmid, B., and Fischer, M. (2007). Genetic isolation of fragmented populations is exacerbated by drift and selection. J. Evol. Biol. 20, 534–542. doi: 10.1111/j.1420-9101.2006.01263.x
Wilson, C. E., Castro, K. L., Thurston, G. B., Sissons, A., and Wilson, C. (2016). Pathway risk analysis of weed seeds in imported grain: a Canadian perspective NeoBiota 30, 49–74. doi: 10.3897/neobiota.30.7502
Zeng, M., de Vries, W., Bonten, L. T. C., Zhu, Q., Hao, T., Liu, X., et al. (2017). Model-based analysis of the long-term effects of fertilization management on cropland soil acidification. Environ. Sci. Technol. 51, 3843–3851. doi: 10.1021/acs.est.6b05491
Keywords: agricultural management practices, agroecosystems, floral scent volatile organic compounds, invasiveness, local adaptation, Pacific Northwest, selection pressure
Citation: Adhikari S, Burke IC, Piaskowski J and Eigenbrode SD (2021) Phenotypic Trait Variation in Populations of a Global Invader Mayweed Chamomile (Anthemis cotula): Implications for Weed Management. Front. Agron. 3:662375. doi: 10.3389/fagro.2021.662375
Received: 01 February 2021; Accepted: 21 April 2021;
Published: 24 May 2021.
Edited by:
Leslie A. Weston, Charles Sturt University, AustraliaReviewed by:
Harminder Pal Singh, Panjab University, IndiaBuddhika Sampath Chamara, University of Sri Jayewardenepura, Sri Lanka
Copyright © 2021 Adhikari, Burke, Piaskowski and Eigenbrode. This is an open-access article distributed under the terms of the Creative Commons Attribution License (CC BY). The use, distribution or reproduction in other forums is permitted, provided the original author(s) and the copyright owner(s) are credited and that the original publication in this journal is cited, in accordance with accepted academic practice. No use, distribution or reproduction is permitted which does not comply with these terms.
*Correspondence: Subodh Adhikari, subodha@uidaho.edu